Life science instruments present unique challenges
to suppliers of light-generation equipment:
Inexpensive,
high-intensity, uniform, monochromatic
light across a broad UV-VIS-IR spectrum is
required. Competition is keen within today’s $50–60
billion biophotonics marketplace1 to find commercial
lighting solutions that provide for all of these
requirements with a low-cost and durable emitter
that, ideally, could be miniaturized for portability.
Today’s commercial light sources include lamps,
lasers, and light-emitting diodes (LEDs). Arc and
tungsten lamps offer white light for a broad range
of uses. Lasers are the brightest and most spectrally
pure light sources, while LEDs offer by far the lowest-cost
light source option. Manufacturers of lighting
equipment have engineered solutions for multicolor
emissions utilizing lamps based on these technologies:
externally filtered lamps, bundled lasers, and
LED arrays. However, no competitive technology
employing these techniques provides a cost-effective
solution for the range of bioanalytical tools used in
today’s life science marketplace.
While each of these solutions has advantages, some
fundamental limitations are not addressed. Lamps
are notorious for their spectral drift and short lifetimes.
Lasers are restricted to particular wavelengths
and require significant safety precautions. LEDs have
limited spectral outputs and although considered an
inexpensive lighting solution, when bundled to generate
adequate intensity, costs associated with their
implementation soar. Currently available commercial
light sources compromise throughput and sensitivity,
lead to frequent instrument calibration, and generate
high cost of ownership. Consequently, instrument
performance is constrained and overly priced.
Based on well-understood light pipe architectures, a
light engine solution based on luminescent pipes has
been developed (Lumencor, Inc., Beaverton, OR) to
fill a void and satisfy the growing bioanalysis market.
Lumencor light engines (patents pending) utilize
solid-state luminescent materials, excitation sources,
and optimized light management techniques. In order
to best appreciate the relative merits of these lighting
tools, a more detailed consideration of the competitive
technical and business landscape follows.
Lamps
The primary light sources for PCR and Sanger
sequencing instruments are tungsten halogen lamps,
arc lamps, and lasers. The tungsten halogen lamp is
a mature product, having been optimized for display
and lighting applications. Improvements in its low
light-collection efficiency or mitigation of high thermal
emissions are unlikely. Durability and stability
issues are also inherent and difficult to resolve. Its
cost could come down, but only if driven by volume
applications. This white light source with a width
of over 1000 nm must be heavily filtered to create
the 10–30 nm bands required in many bioanalytical
instruments. As such, light output to the sample
volume is quite low, on the order of a few hundred
mW/cm2, which relegates use of this lamp to low-throughput
applications.
To overcome this limitation, instrument designs
employ arc lamps. Figure 1 shows the typical emission
curve of a metal halide arc lamp.2 These high-power
lamps generate large light levels. They also
generate very broad white light that must be heavily
filtered. Additionally, they create significant heat
loads that must be managed to avoid compromising
bioanalytical chemistry and instrument performance.
Power instability due to the changing arc over its
lifetime is a notorious problem. Arc lamps have been
significantly improved due to proliferation in newer
microdisplay-based projection televisions and business
projectors. However, the availability of low-cost LEDs
has diffused efforts to improve the arc lamp. Typically
arc lamps, with their associated filters and spectral
wheels, mirrors, controllers, and shutters, add as much
as $10,000 to the cost of a $3000–$5000 bulb.
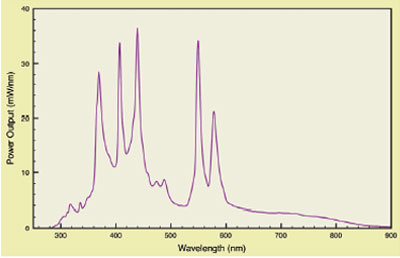
Figure 1 - Typical metal halide arc lamp spectrum.
Lasers
A common alternative to broadband lamps is the
laser. One of the most frequently employed in bioanalyses
is the air-cooled argon ion laser. A typical
laser, for capillary-based sequencing, for example,
provides 10–25 mW in a collimated, multiline beam.
However, the laser color balance is inherently unstable;
the power output of individual wavelengths varies
over time and from product to product. Additionally,
lasers are limited in the number of wavelengths
available, and their speckle patterns are an obstacle
for many applications in which field uniformity is
important. They are nonlinear devices built to be run
in a continuous mode. They are not readily modulated
except by external mechanics. The failure mechanism
of lasers is a catastrophic event. Typical replacement
is required every 6–8 months. Replacement costs are
a function of the wavelength generated and can range
from a few hundred dollars to $10,000, resulting
in high annual maintenance costs. Serious safety
concerns exist about their use by inexperienced
users and in nontraditional testing environments.
As a consequence of the aforementioned shortcomings,
competition from improved light
sources will likely come from diode lasers and
LEDs. Significant improvements in these semiconductor
products have already resulted in
enhanced brightness, increased wavelength range,
and cost reductions. However, diode lasers are primarily
targeted for telecommunications and do not
readily provide wavelengths in the visible and UV
ranges of the spectrum, those required for bioanalyses.
Techniques typically employed to generate
these wavelengths, including frequency doubling,
are unlikely to be employed for low-cost light engine
designs. Difficulties with beam shape, power stability,
and reliability are other issues yet to be resolved.
One exception receiving significant attention in
newer instrument applications is the up-converted
IR diode laser, the so-called diode pumped solid-state laser (DPSS). These lasers are less expensive, more
powerful, and longer-lived than their predecessors.
However, they too demonstrate certain limitations,
namely, a limited number of emission lines and the
need for external modulation, and they remain prohibitively
expensive for many applications.
LEDs
LEDs are now available in a relatively wide range of
wavelengths. However, their output is significantly
broad so as to require filtering to achieve usable bandwidths
on the order of 10–30 nm. Additionally, output
in the visible spectrum is profoundly reduced in
the yellow and green. This gap in usable wavelength
range compromises their utility for bioanalyses where
multicolor excitation throughout the visible spectrum
is required. In many life science applications, sufficient
light intensity cannot be obtained from individual
LEDs, or from arrays of LEDs. Designs based on
LED arrays lead to uniformity, stability, and durability
issues. The spatially broad output results in lower collection
efficiencies and contrast ratios. Their main
advantage, low unit cost, is often offset by the cost
and complexity resulting from additional components
required for their implementation.
Light engines based on light pipes
Most important in the above discussion of traditional
light technologies is that they cannot be readily
improved for bioanalytical applications. The associated
light engine market does not justify the investment
necessary to overcome fundamental performance limitations. As a result, in today’s environment, analytical
instrument performance and price are constrained by
the light source. Moreover, the numerous manufacturers
of lamps and lasers provide only a light source, not
an integrated light engine. The Lumencor light engine
is designed to provide a well-integrated, cost-effective
subsystem that simplifies light subsystem implementation
and does so with enhanced performance.
Light engines are light delivery subsystems that consist
of a light source and delivery optics. A schematic
is shown in Figure 2. Light is generated by exciting
luminescence in a rod or light pipe, which may be
driven by an e-beam, LEDs, or UV bulbs. The light
pipe geometry integrates a significant fraction of the
light, resulting in high external efficiencies. Efficiency
is optimized by the design of the lamp module, including
the excitation source and the geometric shape of
the pipe. Increased power and intensity are obtained
by scaling the light pipe and associated excitation.
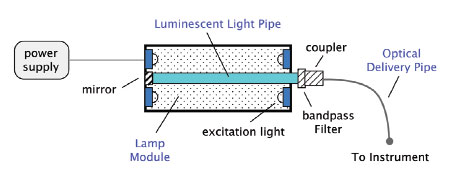
Figure 2 - Schematic of a single-color light engine.
The pipe, which consists of any of a variety of luminescent
materials (e.g., rare earth doped glass, organic
doped plastic, doped single-crystal fiber), is tailored
to emit light in the UV through the IR range. In
the case of the rare earths, emission is inherently
narrow due to their atomic-like energy structure.
Unwanted light is removed with a bandpass filter.
The light output from the luminescent pipe is fed
into an optical delivery pipe and directed into the
analysis instrument or tool. Fast luminescence decay
results in rapid switching, with no appreciable warmup
time for these long-lived, durable materials.3
Each color produced within a light engine requires a
unique combination of light pipe and excitation source.
Moreover, each application requires one of several different
approaches to coupling light from the delivery
pipe into sample volumes. As an example, the light in
a Sanger sequencer is coupled to a row of 50-μm-i.d.
capillaries. Quantitative PCR (Q-PCR) tools typically
require uniform excitation in an array of wells within
a multiple-well plate with no illumination of the interstitial
spaces. Fluorescence microscopes need uniform
light piped through the objective. Microfluidic chips
employ unique detection capillaries and/or flood illumination
of a plurality of channels, spots, or wells. To
satisfy the range of instrument architectures, imaging
and nonimaging optics are utilized.
While no one lighting solution can best satisfy every
instrument architecture, light pipes meet or outperform
the traditional technologies listed in Table 1
on the basis of all figures of merit for all individual
wavelengths. Single outputs, such as red from a diode
laser, may be competitive. However, no family of
outputs can be assembled that bests the pricing of
the light engines described here. As an example, a
Lumencor light engine can emit narrowband light
exceeding 500 mW/color with intensities above 100
W/cm2. Bandwidths as narrow as 10 nm are achievable.
While such output power and overall emission
intensity are impressive, the most significant figure of
merit for quantifying the value of any lighting subsystem
for bioanalytics is the intensity of high-quality
illumination provided to the sample. This is dictated
by the instrument design and sample volume and
is clearly very application specific, e.g., fluorescent
microscopy for live cell imaging vs for reading a chip
designed for gene expression analysis.
Table 1 - General comparison of various sources used in light engines for bioanalyses
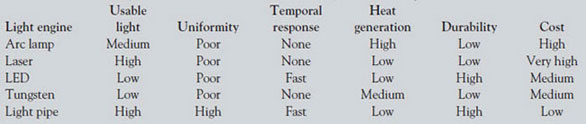
A more detailed comparison of present-day light
engines employed in a number of bioanalytical
instruments with that of a light engine based on light
pipes is shown in Table 2. Significantly, the relevant
comparison is among proprietary lighting subsystems,
not published specifications for a particular
light source. Here, the most important figure of merit
is intensity. The amount of fluorescent signal generated
for a given analyte in each tool is defined by
the S/N of the instrument. This ratio is optimized by
maximizing the number of photons into the analysis
volume and therefore by the illumination intensity
delivered to the analyte in mW/cm2 with the
important caveat that the illumination not degrade
the sample itself. Necessarily, performance is a function
of instrument architecture. Examples include
Sanger sequencers, which use individual argon ion
lasers to simultaneously irradiate an array of up to
96 capillaries (50 μm i.d.) fed electrophoretically
from a multiple-well plate. Tools for Q-PCR most
often require multicolor illumination of high-density
multiple-well plates using mechanical shutters to
switch among wavelengths. Multiplexed chip technologies
and high-density microchannel approaches
are even more demanding in terms of illumination
intensity. Flow cytometry is typically carried out in
serially addressed and spatially separate aliquots of a
continuous flowing, focused stream. Finally, optical microscopes for fluorescence detection require uniform,
stable light, and their intensity requirements
are essentially defined by the objective and the illumination
area under interrogation.
Table 2 - Lumencor light engine output vs light engines based on traditional sources for bioanalytical applications*
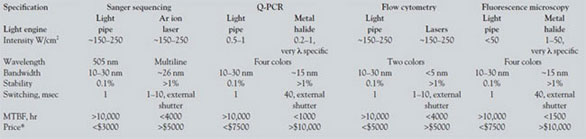
Intensity values differ by orders of magnitude. This is
a direct consequence of the instrument architecture
and the corresponding illumination area dictated by
the sample volume. As a result, highly multiplexed
analysis like those performed using microarrays
require very high intensities to satisfy numerous
small illumination volumes. It should also be noted
that intensity values have a wavelength dependence;
this is much less an issue for light pipes. For example,
while it is possible to achieve W/cm2 intensity values
for a band of light centered at 546 nm out of
the metal halide lamp, much lower intensity values
are produced in other colors such as cyan and red.
Similarly, in the case of light engines based on LEDs,
insufficient power is available between 520 and 590
nm. Relatively, light pipes achieve nonwavelength-dependent
emissions due to the plethora of materials
that are available to produce bands throughout
the UV, VIS, and IR regions of the spectrum; not
every band is possible, but as many as eight unique
colors can be produced with comparable intensity
and brightness. It is possible to integrate the luminescence
for long rod lengths, yielding high emissions.
Finally, because these bands are produced by
individual emitters designed within independently
controllable subunits, light engines have unique
temporal and spatial properties that should enable
new instrument designs and capabilities. Each color
is uniquely operable such that modulation between
colors and simultaneous irradiation by multiple colors
is possible. These light engines make it feasible to
provide controlled excitation for multiplexed analyses
with no external mechanics, filters, or shutters.
Exploitation of this solution for life science applications
promises to enable highly multiplexed analyses
in the next generation of low-cost, portable, high-density
instrument platforms.
References
- Lee, G.; Chu, K.; Conray, L.; Fix, L.; Lui, G.; Truesdell, C. Optik & Photonik June 2007, 2, 30–5.
- www.exfo-xcite.com.
- Bioanalytical instrumentation using a light source subsystem, US-2007-0281322-A1.
Mr. Conner is Vice President, Engineeering; Dr. C. Jaffe is
Vice President, Business Development; and Dr. S. Jaffe is
President, Chief Executive Officer, Lumencor, Inc., 15455
NW Greenbrier Pkwy., Ste. 210, Beaverton, OR 97006,
U.S.A.; tel.: 503-530-1008; fax: 503-536-6741; e-mail:
[email protected].